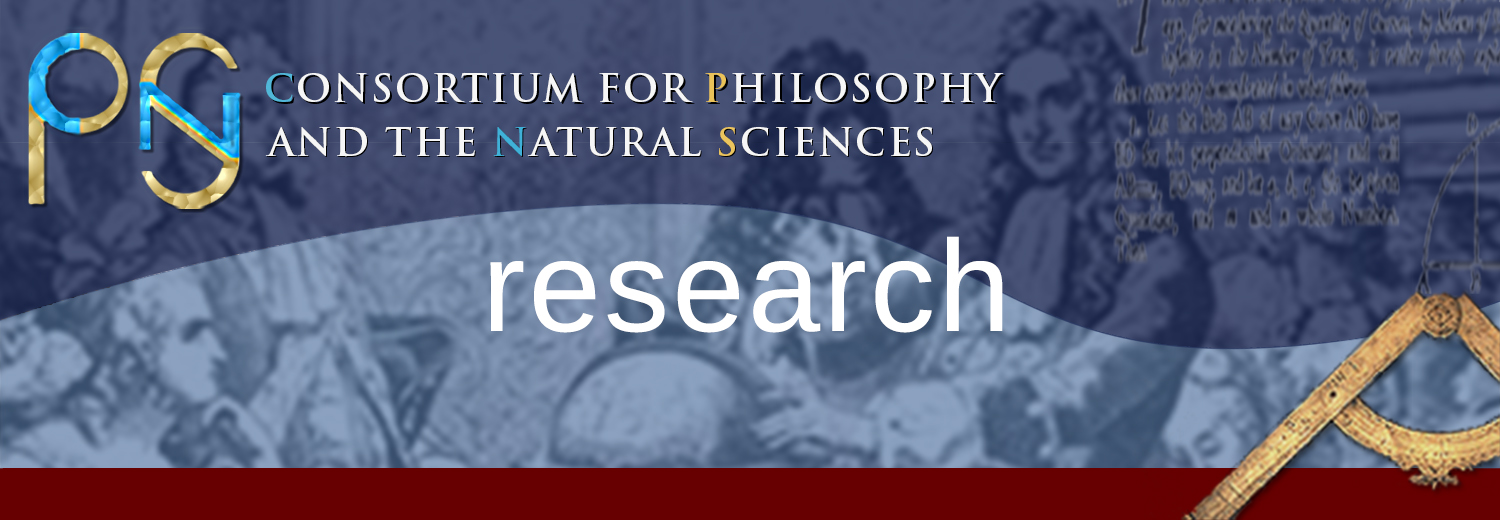
foundations of relational realism
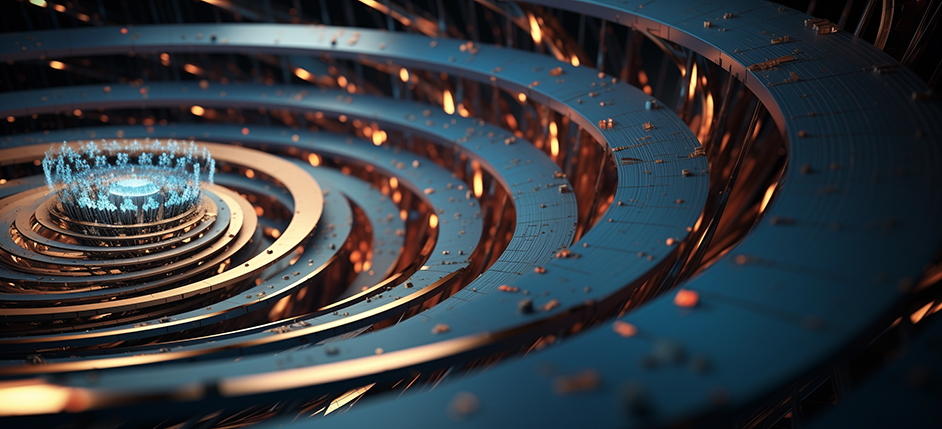
Principal Investigator: Michael Epperson. Co-Investigators: Elias Zafiris, Senior Research Fellow in Theoretical and Mathematical Physics, University of Athens; Stuart Kauffman, Research Professor, Complex Systems Center, University of Vermont; Timothy Eastman, NASA-Goddard; Phillip Stamp, Professor of Physics, University of British Columbia; Karim Bschir, ETH Zurich. Consultants: David Finkelstein, Professor Emeritus of Physics, Georgia Institute of Technology; Roland Omnès, Laboratoire de Physique Théorique Université de Paris XI, (Unité Mixte de Recherche, CNRS). Supported by a grant from the Fetzer-Franklin Fund (Grant D21C62)
This project presents an intuitive, event-ontological interpretation of quantum mechanics formalized via the mathematics of category theory and algebraic topology.
DescriptionExperimentApplications
ARTICLES
Zafiris, E. "The Equiareal Archimedean Synchronization Method of the Quantum Symplectic Phase Space: I. Spinorial Amplitudes, Transition Probability, and Areal Measure of Time" and "II. Circle-Valued Moment Map, Integrality, and Symplectic Abelian Shadows." Foundations of Physics 52, 44 (2022)
Epperson, M. “Relational Realism and the Ontogenetic Universe: Subject, Object, and Ontological Process in Quantum Mechanics.” Angelaki 25:3 (2020)
R. Kastner, S. Kauffman, M. Epperson, "Taking Heisenberg's Potentia Seriously" International Journal of Quantum Foundations, 4:2 (2018) 158-172 (See also Tom Siegfried's review article on this paper in Science News)
M. Epperson, “Event-Ontological Quantum Mechanics: A Process Theoretic Approach” in Physics and Speculative Philosophy: Potentiality and Modern Science. Eds. Timothy Eastman, Michael Epperson, and David Ray Griffin. Berlin: De Gruyter (2016)
E. Zafiris, "The Global Symmetry Group of Quantum Spectral Beams and Geometric Phase Factors," Advances in Mathematical Physics (2015)
Epperson, M. “The Common Sense of Quantum Theory: Exploring the Internal Relational Structure of Self-Organization in Nature,” Coding as Literacy, Metalithicum 5. Ed. Vera Bühlmann and Ludger Hovestadt. Birkhäuser (2015)
E. Zafiris, V. Karakostas, “A Categorial Semantic Representation of Quantum Event Structures,” Foundations of Physics 43 (2013)
K. Bschir, M. Epperson, E. Zafiris, “Decoherence: A View from Topology,” Center for Philosophy and the Natural Sciences, California State University Sacramento (2014)
M. Epperson, “Quantum Mechanics and Relational Realism: Logical Causality and Wavefunction Collapse,” Process Studies, 38:2 (2009)
E. Zafiris, A. Mallios, “The Homological Kähler-De Rham Differential Mechanism, Part I: Application in General Theory of Relativity,” Advances in Mathematical Physics (2011)
E. Zafiris, A. Mallios, “The Homological Kähler-de Rham Differential Mechanism, Part II. Sheaf-Theoretic Localization of Quantum Dynamics,” Advances in Mathematical Physics (2011)
P. Stamp, S. Takahashi, et al. “Decoherence in crystals of quantum molecular magnets.” Nature 476.7358 (2011): 76-79
E. Zafiris, "Boolean information sieves: a local-to-global approach to quantum information," International Journal of General Systems 39, No. 8 (2010): 873–895
M. Epperson, "Relational Realism: The Evolution of Ontology to Praxiology in the Philosophy of Nature." World Futures, 65:1, Routledge (2009): 19-41
M. Epperson, “The Mechanics of Concrescence: Quantum Theory and Process Metaphysics,”
Studia Whiteheadiana, Vol 4 (2010): 159-190
previous projects:
Principal Investigator: Michael Epperson. Co-Investigators: David Finkelstein, Professor, Department of Physics, Georgia Institute of Technology; Henry P. Stapp, Lawrence Berkeley National Laboratory; Timothy Eastman, NASA-Goddard. Click here for Project Description. Supported by a grant from the Fetzer-Franklin Fund (Grant D11C36).